Commercial Roofing as a Sustainability Benefit Multiplier
In other words, if construction waste can be eliminated by recycling, “upcycling,” reusing, or as feedstock for biological systems, the embodied carbon footprint can be minimized or eliminated. This “closed loop” thinking requires a paradigm shift in how we think about industrial products. While it may seem counterintuitive, a shift towards circular thinking can allow us to develop new uses for building materials derived from petrochemicals that have less of an environmental impact than so-called “natural” materials. For example, a recently released study published in the Proceedings of the National Academy of Sciences looking at ethanol biofuel found that, “ethanol is likely at least 24 percent more carbon-intensive than gasoline due to emissions resulting from land use changes to grow corn, along with processing and combustion.”25 This illustrates the importance of a holistic carbon approach using science to overcome our own biases for seemingly counterintuitive truths.
At first glance asphaltic products in roofing may seem like a poor choice from an environmental standpoint. However, when those products are reclaimed at their end of life and recycled into new durable products, the initial embodied carbon is lessened vs. virgin material. In fact, significant strides are already being made by roofing companies that are developing processes to recycle up to 90 percent of torn off asphalt shingles to be used in new shingles or in asphaltic roads.26
In addition, emerging biological technologies like asphalt digesting mycelium are showing promise in new ways to handle petrochemical waste.27 Only by viewing materials from a circular viewpoint can we accurately analyze materials. However, we also need to be careful not to be influenced by savvy greenwashing marketing campaigns and the allure of “wishcycling” as have been commonplace in the plastics industry.28 Only understanding the total whole life carbon footprint of building materials using a science-based approach gives us the clarity to make responsible decisions.
Temporal Considerations of Embodied and Operational Carbon
According to the American Institute of Architects California, “embodied carbon emissions are released during the process that begins with sourcing materials and ends with the completion of construction; operating carbon emissions—from heating, cooling, lighting, and plug loads—occur over the life of a building, which can be 50 years or more. For new buildings, embodied carbon emissions typically equal about 20 years of operating emissions. When looking at total greenhouse gas emissions for new buildings built over the next ten years—the critical period for action to address the global climate emergency—Architecture 2030 estimates that 80 percent will come from embodied emissions, so lowering embodied carbon emissions is now even more urgent than lowering operating emissions” when operational carbon trends toward zero.29
In the short term, embodied carbon emissions have an oversized impact on total emissions. Because the embodied carbon cannot be amortized over the total life of the building (50-75 years) there is not enough time to benefit from the operational carbon savings. However, embodied carbon should not be considered in a vacuum. Operational carbon also needs to be considered for the whole life of the building. Considerations such as product lifespan, durability, and serviceability must also be considered. For example, while it is known that concrete and steel account for the majority of embodied carbon emissions in building construction, until we have better technologies to sequester carbon in materials, create a closed loop circular economy to recycle all building materials, and/or convert all manufacturing source energy to renewable energy, concrete and steel remain the best materials for long-term durability and resilience to more severe and more frequent weather events in a warming climate.
A recent study entitled “Carbon Footprint of Green Roofing: A Case Study from Sri Lankan Construction Industry” by Malka Nadeeshani et al performed a comparative study on a concrete flat roof to a concrete flat roof with a green roof, “The results further revealed that the life cycle carbon emissions of the intensive green roof are 84.71 percent lower compared to the conventional concrete flat roof. Hence, this study concludes that the use of green roofs is a suitable alternative for tropical cities for improving the green environment with substantial potential for carbon emission reduction throughout the life cycle of a building.” And, “The results revealed that the operational phase has the highest contribution to the carbon footprint of both roof types. In the operational phase, the green roof was found to significantly reduce heat transfer by nearly 90 percent compared to the concrete flat roof.”30
By adding vegetative roofs to concrete roof decks, carbon can significantly be reduced. In addition, new approaches to reducing the embodied carbon in materials like concrete are already underway. In April 2021, the UCLA CarbonBuilt project received funding to further study CO2 sequestration in concrete. “The UCLA CarbonBuilt approach captures CO2 generated during production and then infuses it into the concrete, using hydrated lime. The process uses 60 to 90 percent less ordinary portland cement (aka calcium silicate cement), and it doesn’t require as much heat, which has an energy efficiency benefit. CarbonCure also fixes CO2 into concrete. Its equipment (roughly the size of a microwave) is installed for free, but the company using it needs to purchase CO2 elsewhere. Right now, that CO2 is food-grade, but the idea is that it eventually could be collected anywhere.”31
Reducing the embodied carbon of concrete is not some far off future technology. New mixes such as Portland-Limestone Cement (PLC), also known as Type IL, are “engineered with a higher limestone content than portland cement to reduce the carbon footprint of concrete by about 10 percent. It performs just like the cement you’re used to using, resulting in the same concrete you’re used to having. The same specifications, the same mix design, now with a better carbon profile.”32
According to another study, “Using biomass fly ash as a secondary cementitious material can reduce the carbon footprint of concrete by 40 percent while maintaining good technical and environmental performance.”33
Carbon Unification—Whole Life Carbon
Whole life carbon is calculated by adding the embodied carbon emissions to the operational carbon emissions to find the total carbon emissions. In order to analyze whole life carbon, a holistic approach using collaborative design from the very start of the design process is required. As Daniel Overbey has illustrated previously in Building Enclosure magazine, “An ideal whole-building embodied carbon modeling workflow begins at the earliest stages of design as part of an integrative design process.”34
Analyzing commonly used insulation types serves as another example of the balancing act required to properly evaluate whole life carbon. In the chart below is the operational carbon of insulation at increasing thicknesses over 30 years in Climate Zone 5 using gas heat as the primary energy source.
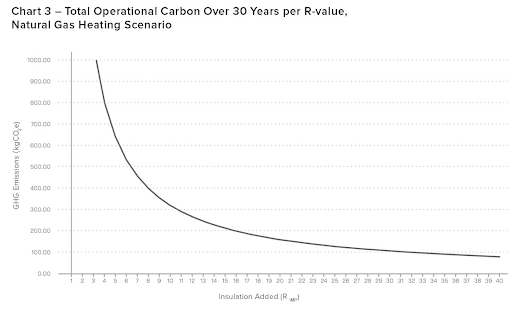
Image courtesy of Canadian Architect magazine
Total Operational Carbon Over 30 Years per R-value in Climate Zone 5, Natural Gas Heating Scenario.
We often hear about the law of diminishing returns of insulation. While it is true that the benefits of adding insulation have less impact on energy savings at a certain point over time, this view is too simplistic. If a holistic performance based approach to the building enclosure is taken in lieu of a prescriptive approach, it is evident that insulation is just one part of an enclosure system. Air tightness, orientation, fenestration performance, thermal bridges, and building occupancy all must be considered in determining the “right-sized” insulation thickness that is specific to the climate where the building resides. Furthermore, the transition to all electric buildings supplied by renewable energy would flatten the operational emissions to zero. However, it should be noted that the building enclosure must still be optimized from an energy efficiency (operational carbon) perspective in order to minimize electric energy consumption and provide the most possible energy back to the grid or battery storage.
Now, keeping in mind what was discussed earlier regarding the oversized short term impact of embodied carbon on whole building carbon, let’s look at the embodied carbon of common insulation types. As different insulation types have different R-values/inch, the best way to standardize the embodied carbon analysis is by R-value. As R-value is directly linked to the energy efficiency of the building, and thereby the operational carbon; evaluating the embodied carbon per R-value gives insight into the interrelationship between the two.
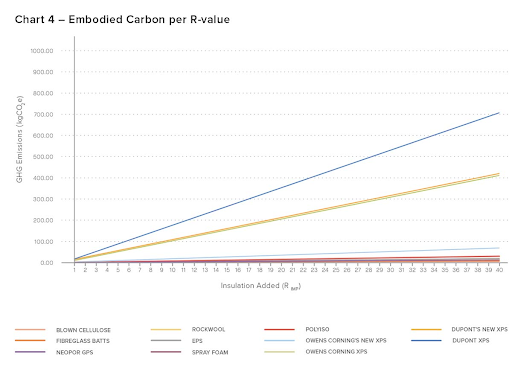
Image courtesy of Canadian Architect magazine
Embodied Carbon per R-value of common insulation types.
By using embodied carbon as the lens to evaluate various insulation types, designers can accurately evaluate the true impact of product selection decisions. Embodied carbon analysis allows us to cut through our own implicit biases and corporate greenwashing to have the greatest immediate impact in reducing up front carbon emissions in the built environment.
Tools for Whole Life Carbon Analysis
While there are a multitude of life cycle assessment (LCA) tools to evaluate the embodied carbon of materials, including Athena, eTool, OneClick LCA, and Tally using EC3 data from the Carbon Leadership Forum (CLF), merging both embodied and operational carbon into one whole building calculator to comprehend the full carbon picture has been an obstacle. However, as reported in Treehugger in January 2022, PHribbon, a new plugin tool for the Passive House Planning Package (PHPP), allows Passive House designers to calculate whole life carbon emissions for Passive House buildings. According to the U.S.-based Passive House Network, "This add-on enables Passive House designers to calculate the embodied carbon of a given design within the Passive House Planning Package (PHPP), an easy-to-use planning tool for energy efficiency. Integrated with the Embodied Carbon in Construction Calculator (EC3), PHribbon gives users unparalleled power to forecast the carbon emissions impact of their designs."35 What is promising about this new tool is that it incorporates all the “in-use” phase embodied carbon emissions into the whole lifecycle of the building, as well as operational carbon by taking into account the use, maintenance, repair, replacement, and refurbishment carbon costs of the building. (See B1-B5 in Figure 19.) In addition, to accommodate future data on the “Beyond Life” aspect of the building materials; reuse, recovery, and recycling potential are also included. (See D in Figure 19.) Only by understanding the interplay of all of these aspects of embodied and operational carbon can we objectively analyze whole life carbon. Whole life carbon must be measured to be managed.
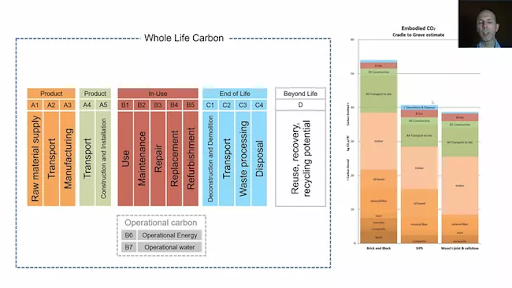
Image courtesy of the Passive House Network
Figure 19. Whole life carbon framework for the PHRibbon in the Passive House Planning Package.
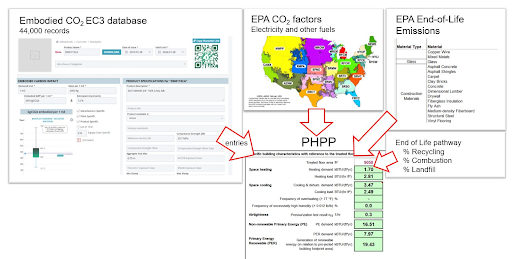
Image courtesy of the Passive House Network
Whole life carbon data integration in PHRibbon for the Passive House Planning Package.
Site Energy vs. Source Energy (Primary Energy)
Understanding the difference between site energy and source energy (or primary energy) is often confusing. Fortunately, through a collaborative effort between ILFI and the Passive House Institute (PHI), a more holistic view is emerging. “Primary Energy (PE) is the total amount of raw fuel that is required to operate a building. In addition to the energy consumed on-site as the building is used, Primary, or Source Energy, includes losses that take place during generation, transmission, and distribution of the energy. PE conversion factors differ for different energy carriers (e.g. electricity or natural gas) and countries. Calculating the PE demand enables an assessment of raw fuel resource needs resulting from building operations based on the energy supply structure.”36
Image courtesy of the Passive House Institute and the International Living Future Institute
Primary energy and site energy.
Furthermore, PHI has a renewable energy conversion factor to calculate how much renewable energy is needed to meet the energy demand of a building.
“To calculate the Primary Energy from Renewables (PER) demand of a building, PER conversion factors are applied as multipliers to the Site Energy. These factors differ depending on the end use (e.g. heating, cooling, hot water), the energy carrier (e.g. electricity or natural gas from renewables), and the region. They take into account storage losses caused by the variability in alignment between regional renewable generation capacity and energy demand for a specific use over the seasons of a year. For generated renewable energy, one kWh is defined as equivalent to one kWh of PER (i.e. PER factor = 1 for renewable energy supply.)”37
Image courtesy of the Passive House Institute and the International Living Future Institute
Electric primary energy from renewable sources.
Overburden for Resilient Design: Integrated Roof Design as a Climate Impact Benefit Multiplier
As defined by the Resilient Design Institute, “Resilience is the capacity to adapt to changing conditions and to maintain or regain functionality and vitality in the face of stress or disturbance. It is the capacity to bounce back after a disturbance or interruption.”38 As illustrated previously, climate change related disasters are increasing in frequency and intensity. Buildings need to be prepared to manage not only the extreme weather related events but also the power outages and the human health and life safety risks that come along with them. Passive energy efficient design strategies ensure that tenants will be able to shelter in place for extended periods without power and not suffer the health-related consequences of extreme heat or extreme cold. By focusing on energy-efficiency first, then adding site renewables and battery storage, tenants may have little interruption to their normal daily activities. Furthermore, if low embodied carbon considerations are included in the energy efficient design, the cause of climate change is simultaneously being addressed.
“Overburden” can be defined as any manner of material, equipment or installation that is situated on top of, and covering all or a portion of, a roof or waterproofing membrane assembly. This excludes thermal insulation but includes, and is not limited to,
- Planters, inclusive of everything they contain;
- Vegetative assemblies in trays, mats or other similar containers;
- Loose growing media, gravel, sand or any other granular material;
- Non-structural water features, inclusive of the water;
- Void fill;
- Tiles, pavers, supporting pedestals or other similar materials;
- Equipment and/or installations, other than those mounted on curbs or structural mounts which are permanently waterproofed with the roof membrane assembly39
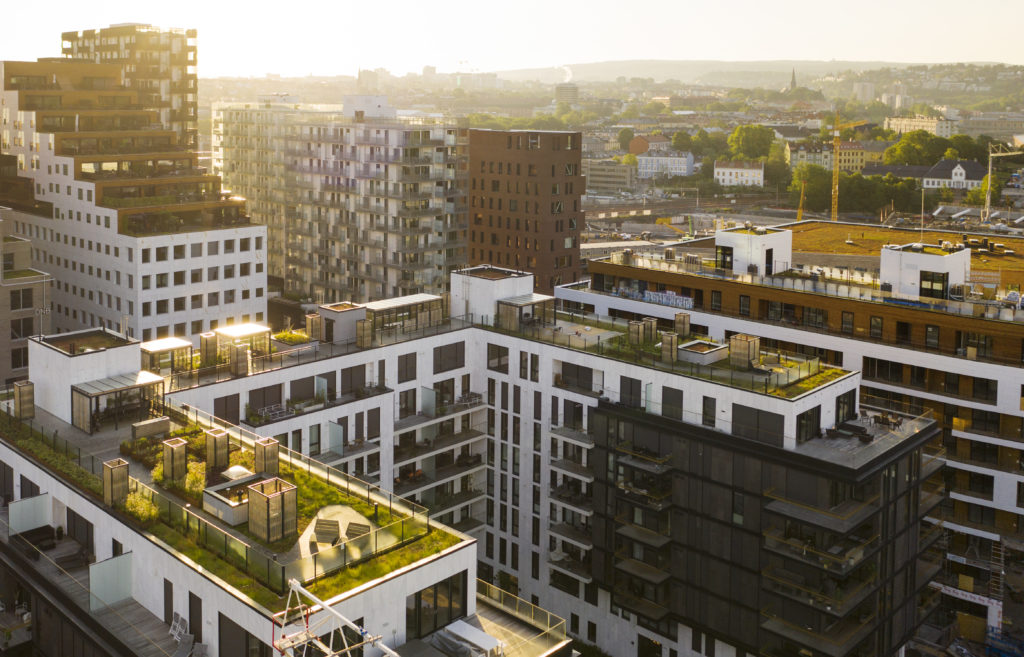
Image courtesy of Standard Industries
Example of overburden.
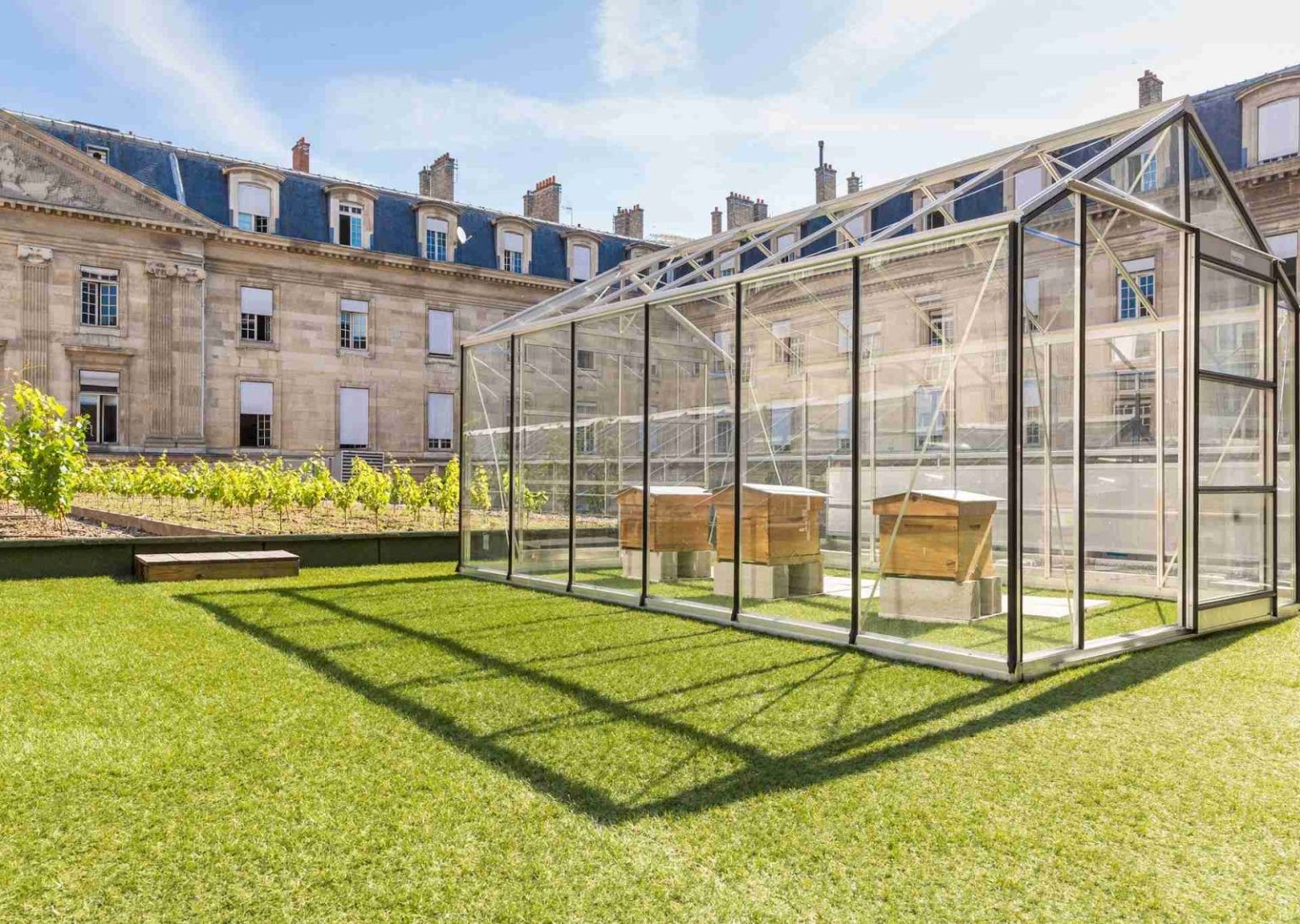
Photo courtesy of Standard Industries
Example of roof overburden with a vineyard, greenhouse and bees.