Stewardship of Glass Products
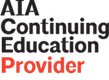
Learning Objectives:
- Explain the concept of stewardship, specifically Environmental, Social and Governance stewardship (ESG).
- Identify the difference between operational carbon and embodied carbon.
- Discuss how operational carbon and embodied carbon are typically measured and reported by glass manufacturers.
- List how the glass manufacturing processes impact embodied carbon content.
- Describe specific methods for reducing operational and embodied carbon through changes to the manufacturing process.
This course is part of the Glass in Architecture Academy
Introduction to Carbon Dioxide
Carbon dioxide is one of several gases in the atmosphere called greenhouse gases (GHG)—that trap heat and contribute to some of the Earth’s temperature increases. Other GHGs include methane, nitrous oxide, and fluorinated gases. But carbon dioxide accounts for more than 79% of all GHG emissions emitted by the U.S. in 2021, according to the Environmental Protection Agency.3
Activities that emit carbon dioxide include burning solid waste, trees, and other biological materials, and burning fossil fuels including coal, natural gas, and oil. It’s also a byproduct of some chemical processes, including cement production. Carbon dioxide is naturally removed from the atmosphere when it’s absorbed by plants through photosynthesis.
Governments at all levels are starting to set goals and develop policies designed to reduce greenhouse gas emissions associated with the built environment. For example, Local Law 97 in New York City sets energy efficiency and greenhouse gas emissions limits on most buildings over 25,000 square feet.
At the state level, the Buy Clean California Act and the Buy Clean Colorado Act are U.S. examples of set maximum embodied carbon (global warming potential) limits for certain categories of construction materials.
At the federal level, the U.S. General Services Administration (GSA) in May of 2023 announced a six-month program to pilot interim requirements for the use of low embodied carbon construction materials in GSA projects funded by the Inflation Reduction Act of 2022.4 In December of the same year, the final requirements were published.5 These requirements set global warming potential limits for asphalt, concrete, float glass, and steel in support of the Federal Buy Clean Initiative, which promotes the use of lower carbon construction materials made in the U.S.6
In other parts of the world, governments have been advancing energy codes to reduce their nations’ overall energy use.
Designers and architects can explore methods to lower carbon emissions through both the embodied and operational carbon avenues. Often, they will organically dovetail together.
Embodied Carbon
Embodied carbon is often considered from the following perspectives, depending on the life cycle stages in question: cradle-to-gate, cradle-to-grave, and cradle-to-cradle.
From a cradle-to-gate perspective, embodied carbon refers to the carbon dioxide equivalent associated with the extraction, processing, transport, and manufacturing of a product, product family, or service.
The cradle-to-grave framework in the building industry includes everything in the cradle-to-gate time frame plus construction, maintenance, repair, and end-of-life stages.
The cradle-to-cradle perspective expands the concept of the cradle-to-grave framework to include options for material reuse and recycling.
Operational Carbon
Operational carbon refers to the amount of carbon emitted while a building is in use through day-to-day activities like lighting and operating its HVAC system—heating, venting and air conditioning–as well as the daily activities of occupants in the building.
An energy grid that relies on renewable sources rather than traditional coal or natural gas power generation can help reduce the impact of operational carbon output. Over the last several decades, new and innovative design techniques along with technology advancements have helped buildings become more energy efficient. Buildings are using less energy overall and, in many areas, the grids supplying the energy have a higher percentage of renewable sources (e.g. solar panels and wind turbines), which contributes to the reduction of the carbon emissions associated with energy generation.
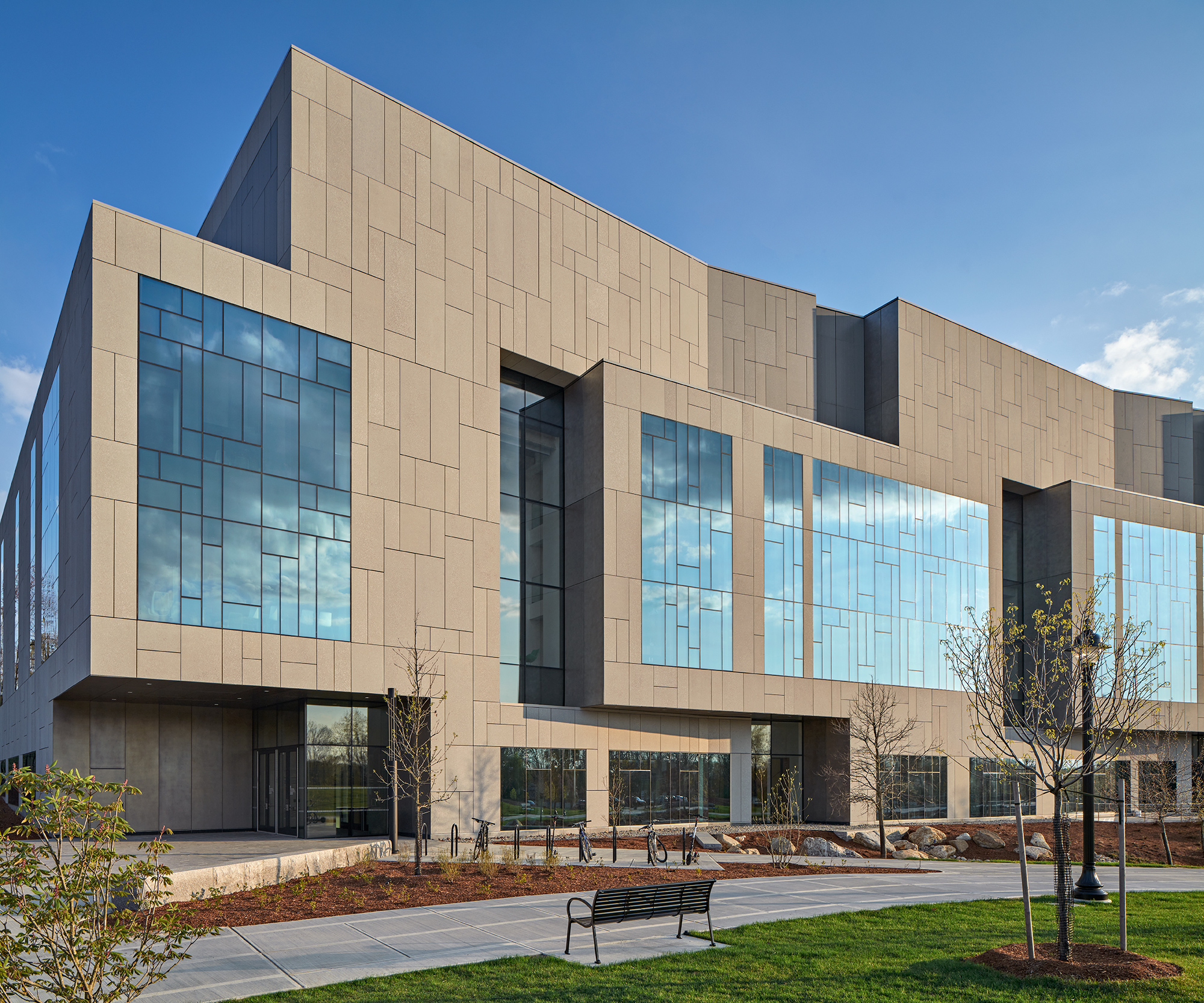
Photo by Robert Benson Photography; courtesy of Guardian Glass
Science 1 Research Center is a three-story, high-intensity academic research building dedicated to the interdisciplinary fields of material science and engineering at the University of Connecticut in Storrs, Conn. High-performance, low-E coated glass helped the project achieve LEED Gold status.
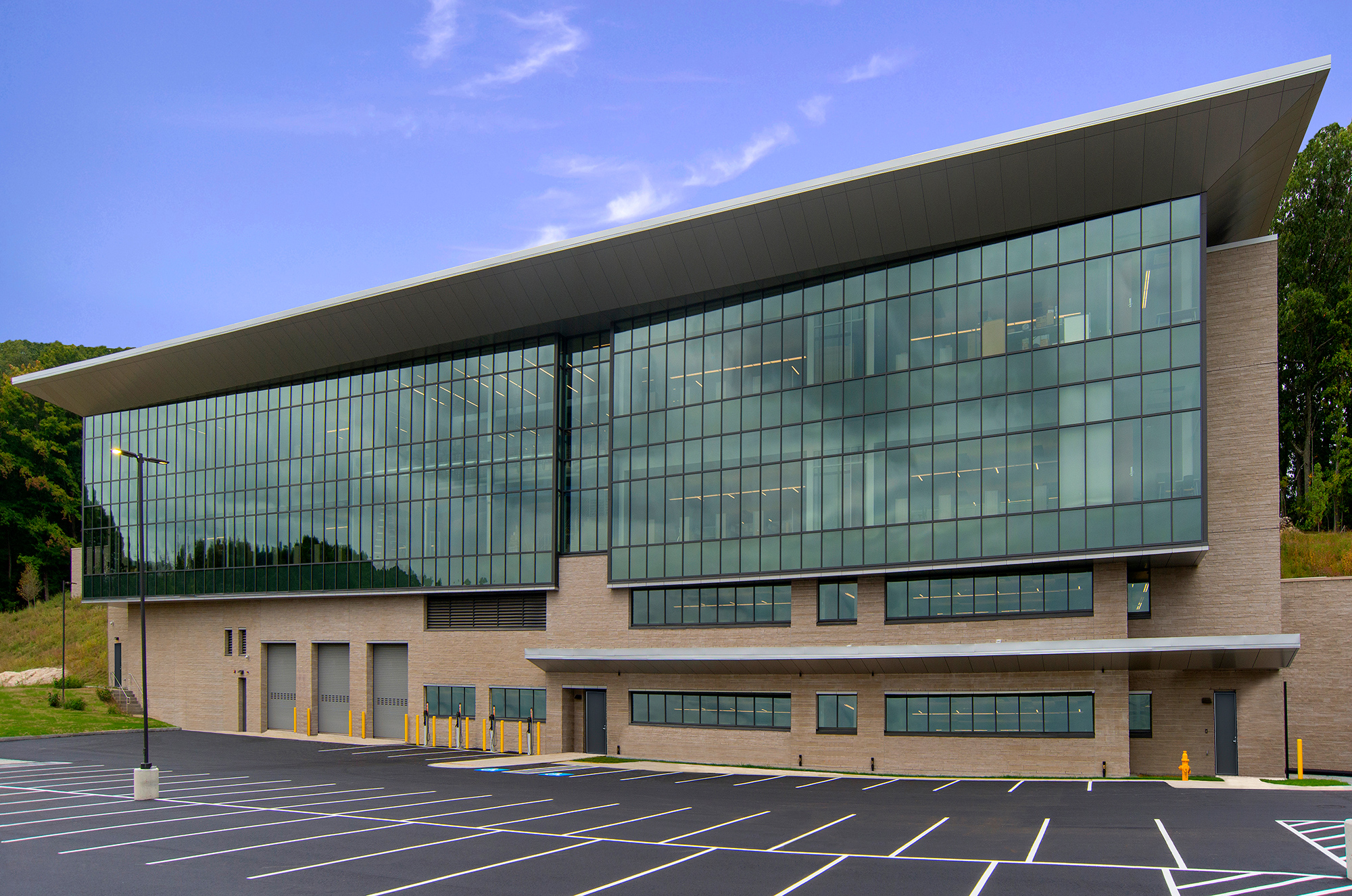
Photo by Casey McNamara; courtesy of Guardian Glass
Designed as a Net-Zero-Energy Building, the Massachusetts Department of Transportation building in Worcester, Mass. incorporates glass extensively into the exterior to allow ample natural daylighting and a modern design.
Life-Cycle Assessments of Buildings
As the name suggests, a Life-Cycle Assessment (LCA) is a way to understand the environmental attributes of a product, product family, service or even building by breaking down attributes by life stages: resources used, processing, manufacturing, distribution, the use of the completed building, and the end-of-life management.
When looking at an LCA for a building, the first stage is materials. This involves assessing the sources and quantities of the materials used in a project and how they are made, following that process from the mine to the construction site.
Next is the building process. For example, how much carbon does the construction process release into the atmosphere? This involves looking at the inputs and outputs of the construction process: energy used, waste produced, and emissions released during the building phase.
The next phase of the life cycle is the use of the completed building. This phase includes use of energy for heating and cooling, for systems like HVAC, for lighting the building, and any other day-to-day operations, as well as maintenance.
The final phase is a building’s end of life stage. What happens once the building is no longer being used? Will it be demolished? Can parts of it be recycled or reused?
The goal of an LCA is to help builders and designers understand the environmental attributes of their projects, and minimize them where possible and feasible, by the design choices made (materials, sourcing, quantities, transportation). LCAs can help you verify you’re moving towards your project or company’s environmental target. While making the LCA of a whole building needs specific knowledge and can bring complexity, collecting and reviewing independent third-party verified EPDs of the main materials selected can point you in the right direction to evaluate the embodied and operational carbon of a building.