Designing Modern Wood Schools
How to create high-performance structures that are also cost effective
Continuing Education
Use the following learning objectives to focus your study while reading this month’s Continuing Education article.
Learning Objectives - After reading this article, you will be able to:
- Review provisions of the International Building Code specific to school buildings and discuss opportunities to achieve cost savings through the use of wood.
- Explore design and detailing best practices used to achieve performance objectives in school assembly design.
- Discuss structural design considerations unique to school buildings, as well as framing options for floors, walls, and roofs.
- Consider how wood has been used in modern wood-frame and mass timber schools across the United States.
There is a strong case to be made for using wood in school construction, both to accommodate a growing number of students with structures that are cost effective, and to do so while creating high-performance buildings that are safe, resilient, and appealing.
Across the United States, there is high demand for new schools. In 2019, an estimated $98 billion was spent on educational construction, including new buildings and structures as well as renovations, site work and all associated utility work. School construction, and educational facilities accounted for about 101 million square feet of the nonresidential market.1 Since, by 2024, U.S. schools will be required to accommodate an estimated 2.8 million more students than they do today, these numbers can only increase.2
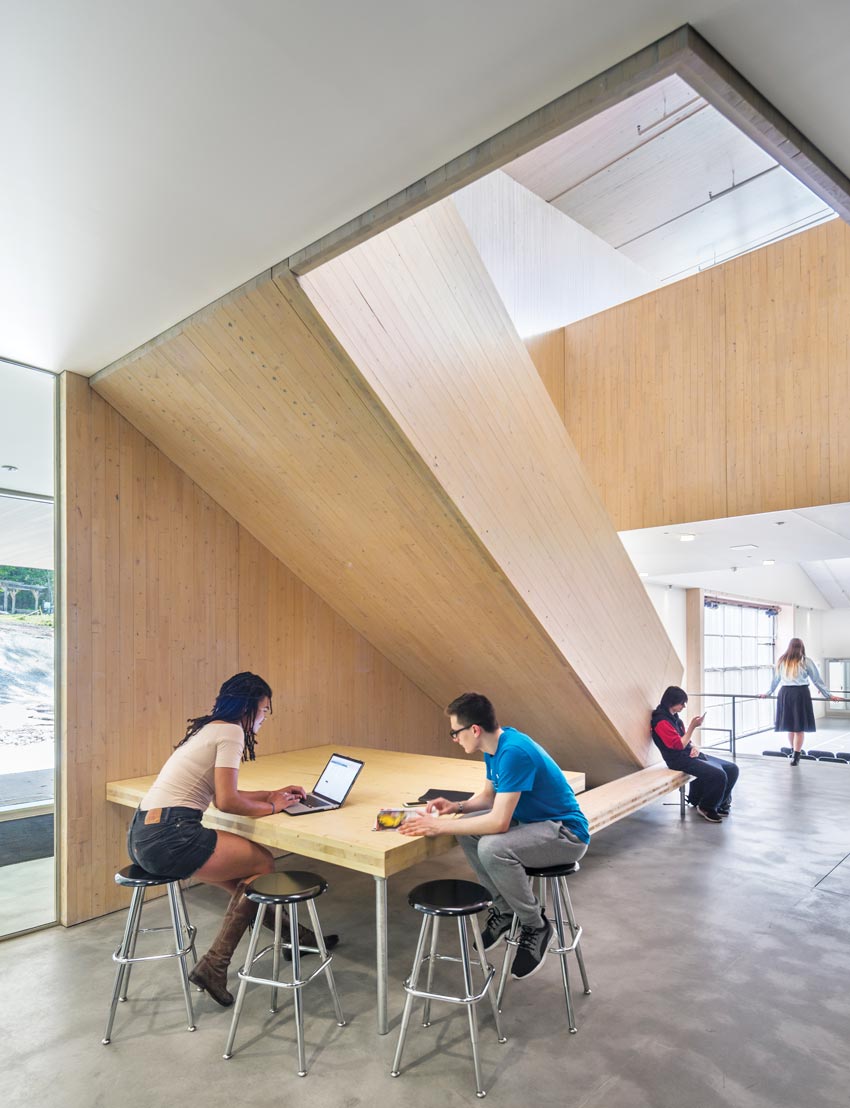
Photo: David Sundberg
Location: New Haven, Connecticut
Architect: Gray Organschi Architecture
Timber Engineer: Bensonwood
Exemplifying the trend toward mass timber in school design, this 14,000-square-foot addition to a Type VB high school is comprised of CLT and glulam.
Cost and construction speed are often cited as the main reasons to design a school in wood. Wood building systems typically cost less than alternatives, and wood construction is fast, even more so with the trend toward panelized products, such as cross-laminated timber (CLT), and prefabrication. This is especially important for schools, which often have limited budgets and compressed construction schedules.
Increasingly, however, school designers and facility planners are citing other attributes of wood as motivating factors for its use. They point to its light carbon footprint, energy performance, and other environmental benefits. They also cite a growing body of research linking the use of exposed wood to occupant well-being, including potential benefits related to increased concentration. In school construction, wood offers endless opportunities to create warm and inspiring places to learn.
This course takes a practical look at the design of wood schools, emphasizing opportunities with traditional wood-frame construction and, in particular, how to reduce costs. Architectural design and detailing topics include allowable heights and areas, detailing for fire resistance, acoustics, and durability, as well as structural design considerations. The trend toward mass timber is also discussed, along with information on wood’s biophilic attributes and environmental performance, including energy efficiency and carbon footprint. Examples of wood schools across the United States are also highlighted.
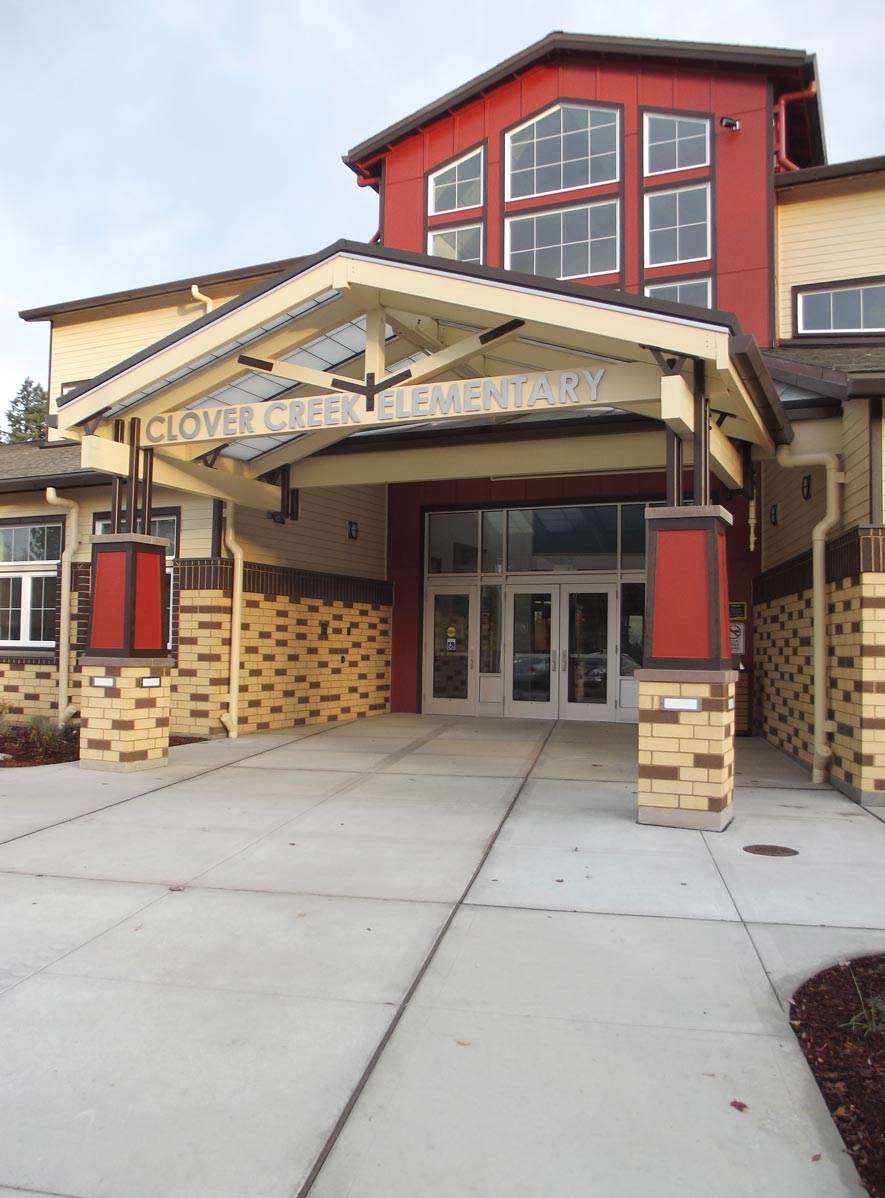
Photo: Bethel School District
Location: Tacoma, Washington
Architect: Erickson McGovern Architects
Structural Engineer: PCS Structural Solutions
Bethel School District uses Type VB Construction to save upfront construction costs and super insulates to reduce utility costs. It uses the savings from both to buy more energy-efficient but expensive mechanical and lighting systems, which further add to the savings. Director of Construction and Planning Emeritus Jim Hansen, says, “We need people to believe we do a good job, not only educating their children but managing their money.”
Construction Type and Cost
In Washington state, the Bethel School District’s strategy is to save money by using wood-frame construction for the majority of school construction, and to use those savings to buy more expensive but efficient mechanical or lighting systems. This provides operational savings—most of its schools are ENERGY STAR leaders—which, over the long term, puts less pressure on the general fund. The district reports construction costs per square foot that are much lower than the average in the region, an achievement Director of Construction and Planning Emeritus Jim Hansen credits to the use of wood.3
On a national basis, in 2019 construction costs for primary and secondary education facilities ranged from $230 per square foot in Tennessee to $558 per SF in New York. According to the "State of School Construction, 2015 Report" by School Planning & Management, average school sizes are:
- Elementary schools: 80,000 square feet
- Middle schools: 117, 000 square feet
- High schools: 154,700 square feet
The majority of schools are one or two stories (Figure 1), and relatively few are built in wood. Rather, many designers default to steel or concrete, even though wood schools are permitted under the International Building Code (IBC), are required to meet all of the same safety and performance requirements as schools built with other materials, and can offer significant cost savings.
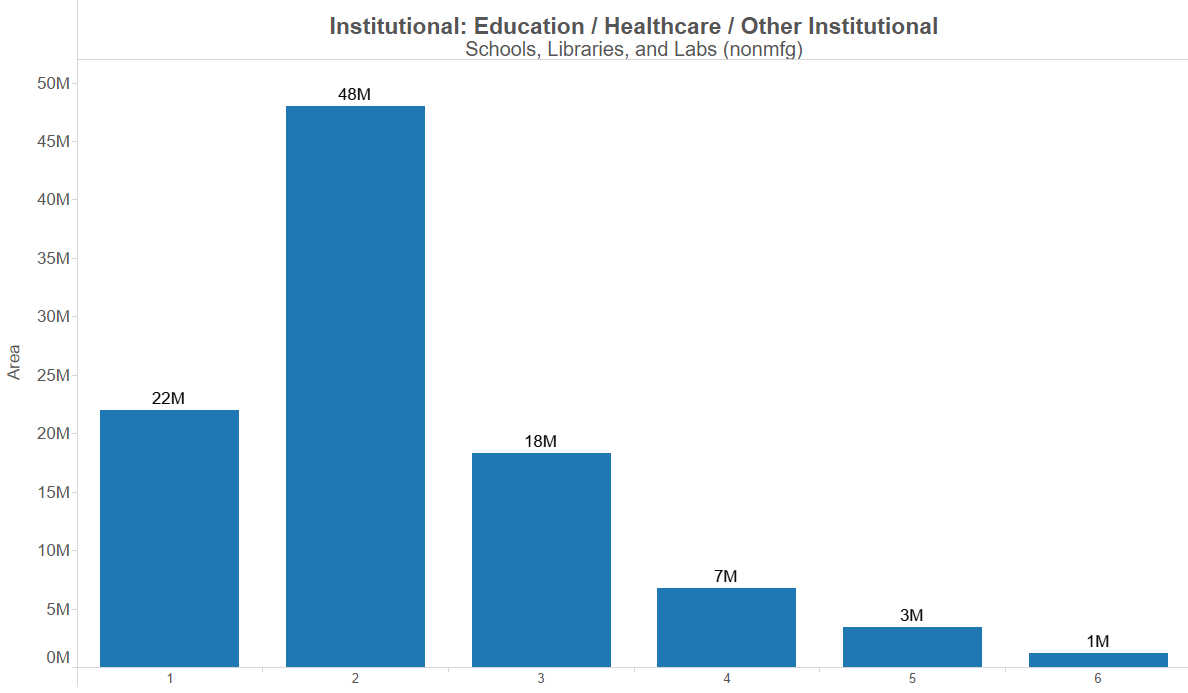
Source: Dodge Construction Data, 2019
Under the IBC, small and medium-sized spaces in a school typically fall under Educational Group E occupancy. Although large spaces such as a gymnasium or cafeteria can be classified as Assembly Group A, IBC Section 303.1.3 allows schools to be classified as Group E throughout, and this is a common approach.
IBC Section 602 defines five construction types and allows the use of wood as follows:
- Types IIIA, IIIB, IV, VA, and VB: Structural wood framing permitted throughout
- Types IIIA, IIIB, and IV: Fire retardant-treated (FRT) wood framing required for exterior walls
- Type IV: Exposed heavy timber permitted for interior elements provided they meet the minimum size requirements of IBC Section 602.4
- Types IA, IB, IIA, and IIB: Several provisions for the use of wood per IBC Section 603
The IBC specifies the allowable height and area for each construction type, and each has different requirements, largely related to fire protection.
Twice a year, the International Code Council (ICC) publishes building valuation data that includes the average cost per square foot for each construction type and occupancy group. Figure 2 shows the average cost of buildings in Educational Group E, and illustrates the cost impact of construction type and, by extension, choice of building material. Buildings of Type I and II Construction, which are typically steel, concrete, or masonry, cost an average of $188 to $210 per square foot. Buildings of Type III and V Construction, which are typically wood-frame, cost significantly less at $149 to $175 per square foot.
Note: The ICC data includes building costs only (e.g., foundation, structure, mechanical), while the School Planning & Management report cited above includes complete project costs (e.g., furnishings and site work).
Given the potential savings, the question becomes: Is it possible to design an average size school—i.e., 80,000 to 155,000 square feet—as a Type III or V wood building? The answer is yes. Although designers accustomed to steel and concrete often design schools as Type IIA or IIB, nearly identical height and area can be achieved with wood framing (Figure 3).

Source: ICC Building Valuation Data, February 2020
The ICC publishes cost per square foot averages by occupancy group and construction type.

Source: IBC Tables 504.3, 504.4 and 506.2
With Type III Construction, a wood-frame building can achieve almost the same height and area as a steel or concrete building of Type II Construction. Designers can then use code provisions for further increases.
Code Provisions for Height and Area Increases
For all but Type I buildings, the square footage shown in Figure 3 is clearly much less than the average school sizes stated above. These are base heights and areas, and numerous code provisions exist for increases beyond those amounts. In the context of a wood-frame school, for example, designers may utilize the following:
Sprinklers: The requirement to include an NFPA 13 sprinkler system is not based on materials or construction type. It is based on occupancy group, occupant load, size of the fire area, and other occupant-specific criteria. Per IBC Section 903.2, an NFPA 13 sprinkler system is required throughout all educational and assembly occupancies where the fire area exceeds 12,000 square feet—which includes the vast majority of school construction. Use of an NFPA 13 sprinkler system allows designers to significantly increase the height and area of these facilities.
- Per IBC Tables 504.3 and 504.4, buildings equipped throughout with an NFPA 13 sprinkler system can add one story and 20 feet to the base stories and heights in IBC Table 503.
- Per IBC Table 506.2 and Section 506.2, buildings equipped throughout with an NFPA 13 sprinkler system can add 200–300 percent to the base floor areas in Table 503. For a single-story building, the base area can be multiplied by four. For a multistory building, the base area can be multiplied by three.
- The story and height increases are permitted to be used concurrently with the area increase per IBC Tables 504.3, 504.4 and 506.2.
Open frontage: Open space around a building, such as a parking lot or major roadway, provides firefighting access to multiple sides of the structure. If more than 25 percent of the building’s perimeter is open for a minimum of 20 feet, IBC Section 506.3 allows an increase to the unsprinklered floor area in Table 506.2 of up to 75 percent.
The area increases for sprinklers and open frontage are per story. Per IBC Section 506.2.3, a two-story school building’s total area is permitted to be twice the increased area, and a three-story (or taller) school building’s total area is permitted to be three times the increased area. Figure 4 shows the total potential square footage impact for Educational Group E occupancy.
Compared to a Type IIA steel or concrete building, a Type IIIA wood-frame building has the same height limits of four stories and 85 feet. The Type IIIA building offers nearly 90,000 square feet per floor, and more than 176,000 square feet for a two-story building. Even a Type VA structure offers an area of nearly 139,000 square feet for a two-story building, which is larger than many schools.
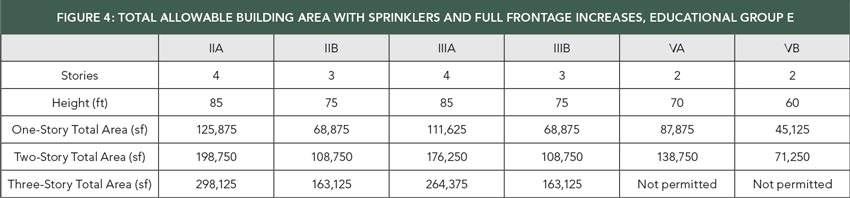
Source: IBC Tables 504.3, 504.4 and 506.2
With sprinklers and open frontage, Type III and Type V Construction offer ample height and area for most schools.
Unlimited area: Per IBC Section 507.11, a wood-frame school may also qualify for unlimited area—if, for example, the building:
- Has a minimum of 60 feet open frontage around the perimeter
- Is Type IIIA or IV Construction, one story, fully sprinklered, and classified as Educational Group E occupancy
- Is Type III or IV Construction, one story, fully sprinklered, and classified as Assembly Group occupancy (A-4)
- Meets other provisions such as those related to egress
Fire walls: Fire walls are the most restrictive type of wall assembly in terms of their construction and hourly fire-rating requirements. However, they allow areas within a structure to be considered as separate buildings for the purpose of calculating height and area, further increasing the potential size of a project.
The Savings Add Up
Combine the ICC’s estimated cost per square footage from Figure 2 with the average school size and costs from the School Planning & Management report, and a picture emerges of significant cost savings. By switching from a Type IIA steel or concrete school to a Type IIIA wood-frame school, the ICC estimates show a potential 11 percent savings. Now factor in the average school size:
- Elementary school: 80,000 square feet/save $1.7 million
- Middle school: 117,000 square feet/save $2.5 million
- High school: 154,700 square feet/save $3.3 million
For most elementary and middle schools, the average size falls within the maximum allowable by Type VA Construction, bringing the potential savings closer to 22 percent.
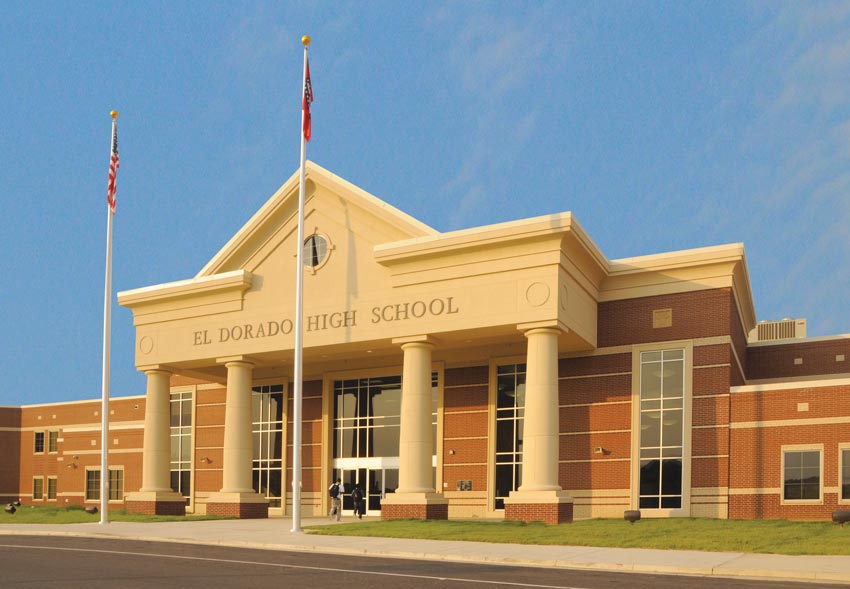
Photo: Dennis Ivy; courtesy of WoodWorks
Location: El Dorado, Arkansas
Architect: CADM Architecture
Structural Engineer: Engineering Consultants, Inc.
When the initial steel and masonry design for this 320,000-square-foot, Type IIIA high school came in well over budget, the project team evaluated alternative systems. The intent had always been to utilize exposed heavy timber in select areas. However, by changing approximately 40 percent of the nonexposed structural materials to wood framing, the team was able to save $2.7 million.4
Detailing for Fire Resistance
An important yet little known piece of information for many designers is that there are many sources for tested assemblies that meet 1-hour and 2-hour fire-resistance ratings required for wood buildings—not just UL.
In addition to UL’s Fire Resistance Directory, assemblies can be found in publications such as:
- Intertek Testing Services’ Directory of Listed Products
- Gypsum Association’s Fire Resistance Design Manual
They can also be selected from one of the prescriptive assemblies provided in IBC Section 721, which are based on ASTM E 119 or UL 263 test results, by calculating an assembly’s fire resistance using IBC Section 722, or by other methods indicated in Section 703.3 of the code.
Assemblies tested by the wood industry are also available. AWC’s Design for Code Acceptance 3: Fire-Rated Wood Floor and Wall Assemblies contains fire ratings of wood-frame wall and floor/ceiling/roof assemblies. Other sources include APA – The Engineered Wood Association’s Fire-Rated Systems (Form W305), and Wood Truss Council of America’s Metal Plate Connected Wood Truss Handbook. Some manufacturer websites and catalogues also reference tested assemblies that include their products.
Designers also have the option of integrating exposed, fire resistance-rated heavy or mass timber structural members into their designs, adding warmth to interior spaces. Because these products are thick and solid, they char on the outside at a slow and predictable rate, while retaining strength, slowing combustion, and allowing time to evacuate the building. The char protects the wood from further degradation, helping to maintain the building’s structural integrity and reducing its fuel contribution to the fire.
Per IBC Section 722, the fire resistance of exposed wood members may be calculated using the provisions of Chapter 16 of the NDS. AWC’s Technical Report No. 10: Calculating the Fire Resistance of Exposed Wood Members, contains full details of the NDS method as well as design examples.
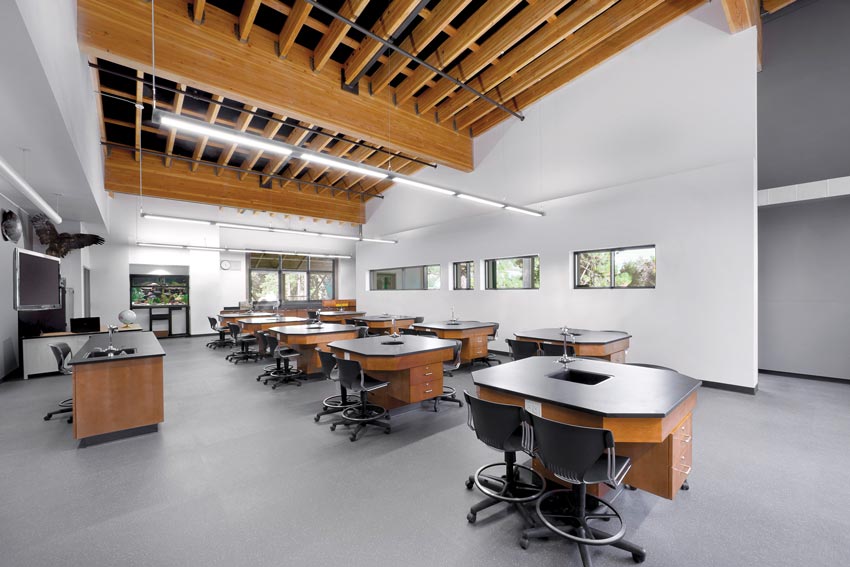
Photo: Costea; courtesy of LPA, Inc.
Location: South Lake Tahoe, California
Architect: LPA, Inc.
Structural Engineer: LPA, Inc.
Size: 67,500 square feet
Fire resistance of exposed wood members can be calculated using the provisions of Chapter 16 of the NDS.
Acoustics
With spaces that vary from gyms to libraries (and every noise level in between), acoustic consideration is an obvious priority for school design. The IBC divides sound into two categories. Airborne sound is measured with sound transmission class (STC) ratings and is relevant both to wall and floor/ceiling assemblies. Structure-borne sound is measured through impact insulation class (IIC) ratings and only relates to floor/ceiling assemblies.
While the IBC requires STC and IIC ratings of 50 for assemblies in apartment buildings and hotels, it has no such requirements for educational facilities. However, many school districts have established their own minimum ratings, often with similar STC and IIC baselines.
Tested wood-frame assemblies are available to meet a wide variety of acoustic performance levels. This is illustrated in Figure 6, which shows the progression from single-stud through staggered stud and double-stud construction. Double-stud walls can achieve a rating of approximately STC 63 when insulated with batt insulation and covered with two layers of gypsum wallboard on the outside faces of the studs.
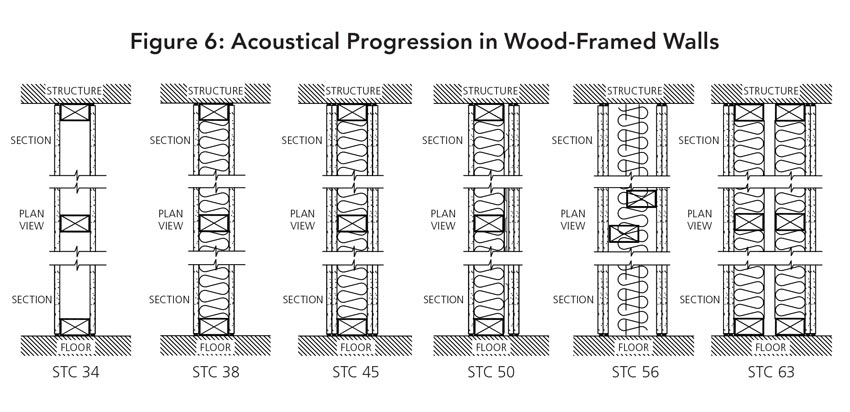
Source: Acoustical Considerations for Mixed-Use Wood-Frame Buildings, WoodWorks
Beyond gypsum wallboard and insulation, options for improving performance include (among others) resilient channels in walls and floors, and concrete topping (or other similar material) on floor assemblies. For a more in-depth discussion of acoustic detailing, the WoodWorks paper, Acoustical Considerations for Mixed-Use Wood-Frame Buildings, is also relevant to the design of wood-frame schools.5
For information on the acoustical design of mass timber floor and wall assemblies, as well as a list of tested assemblies, see the WoodWorks paper Acoustics and Mass Timber: Room-to-Room Noise Control and its accompanying Inventory of Acoustically-Tested Mass Timber Assemblies.
Durability
There is a misperception that wood buildings require greater levels of maintenance than those made from other materials or don’t last as long, and architects have cited this perceived limitation as a particular issue for schools. However, with proper design and detailing, wood schools can match the durability performance of schools made from any other material.
In the context of durability, there are two main concerns: areas of high traffic and high moisture.
In high-traffic areas, the structural material doesn’t tend to be at risk unless the structure is also the finish material, as it often is with a CLT or other mass timber school. Common options for avoiding damage include high-durability finishes, such as hard tile, medium-density fiberboard, impact-resistant gypsum, and vinyl wall coverings. To make these finishes cost-effective, they are often added just to the lower portion of the wall (e.g., the bottom 6 feet) where the most wear and tear can be expected.
In high-moisture areas such as bathrooms and labs, it is useful to both use durable finish materials and elevate the wall structure and finishes off the floor by installing a curb below the walls. See the WoodWorks publication Wood-Frame Schools: Durability Techniques for Interior High Traffic and Moisture Areas https://www.woodworks.org/wp-content/uploads/Wood-Frame-Schools-Durability-Techniques-WoodWorks.pdf.
For information on durability detailing related to the building envelope, including moisture, fungi, and termite control, the Architectural Record CEU, “Designing for Durability,” is available at www.ThinkWood.com.
Structural Design
Schools offer unique design challenges, in part because of the great variety of spaces. Requirements include a mix of smaller spaces such as classrooms, offices, corridors, and bathrooms; medium spaces such as choir rooms and labs; and large spaces such as gyms, cafeterias, and libraries.
Although a detailed discussion of structural design options is beyond the scope of this course, this section will consider typical school requirements and demonstrate how they can be met with wood-frame construction, while at the same time reducing costs.
Many schools include long, rectangular classroom wings, separated from the gym and cafeteria, with classes that feed into a corridor from both sides. Classrooms are typically 800 to 1,100 square feet, sized to accommodate 20 to 30 students, and are square to slightly rectangular. Common classroom sizes include 28 by 30 feet, 30 by 30 feet, and 32 by 32 feet, while corridors tend to be 6 to 18 feet wide. Minimum ceiling height is typically 9 feet, with a floor-to-floor height of about 13 feet.
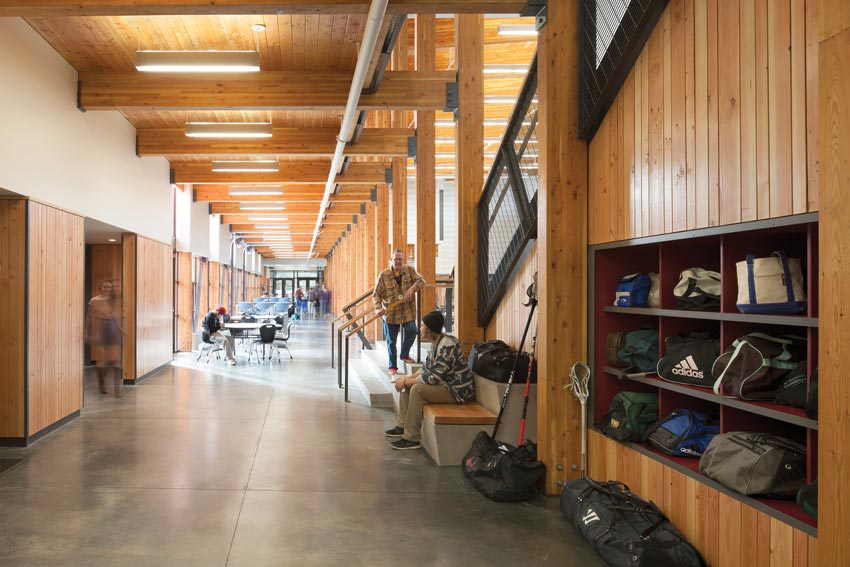
Photo: Lara Swimmer
Location: Vashon Island, Washington
Architect and Engineer: Integrus Architecture
Typical school corridors range from 6 to 18 feet. At this 84,000-square-foot, Type V school, dimension lumber, glulam, and light-frame trusses were used for the structure, reflecting the community’s values and desire to promote thoughtful stewardship of natural resources.
Structural Loads
An important aspect of the IBC is that it is scaled to reflect risk. Per IBC Table 1604.5, buildings are classified into risk categories based on use, from Risk Category I for those representing a low hazard to human life in the event of failure (such as storage buildings) to Risk Category IV for structures with greater consequences associated with their failure (such as hospitals). They are further defined based on the likelihood of a specific type of event occurring. Buildings constructed in regions known for hazards, such as hurricanes, earthquakes, or floods, are subject to design requirements that make them better able to withstand these events.
Educational facilities are generally Risk Category III and must be designed for structural loads that are 10 to 25 percent higher than buildings in lower risk categories. Common loadings include:
- Classroom floor live load = 40 pounds per square foot (psf)
- Corridor floor live load = 80 to 100 psf
This is where the relative light weight of wood-frame systems can be a cost advantage. Even a floor system detailed to meet objectives for fire resistance and acoustics—with gypsum wallboard, lightweight concrete topping, resilient channels, and insulation—results in a floor dead load of just 25 to 35 psf. For comparison, a structural steel system with cast-in-place concrete or a precast concrete slab would likely be twice that amount.7 Wood’s light weight also has potential benefits in terms of foundation and seismic requirements (seismic force is relative to weight), which add to the savings.
It should be noted that the IBC allows reduced loads in certain cases that are relevant to school design. Where members are used to support a large surface of floor area, the code recognizes the unlikelihood that the entire area will be loaded to its maximum all the time. Per ASCE 7-16: Minimum Design Loads for Buildings and Other Structures, Section 4.7.2, exterior and interior columns and beams in spaces that meet minimum tributary area requirements may be designed for lower live loads. In a 32-by-32-foot classroom, for example, a column in the exterior wall can be designed for 23 psf live load instead of the standard 40 psf.
Floor Framing
Wood framing is a viable choice for floor spans of 25 to 32 feet, which are typical of classrooms. It can meet the same safety and performance requirements, and follow essentially the same grid design as other materials, but often at much less cost. While savings vary by city, cost estimates performed by EQS Consultants based on RS Means data for 2016 Q2 indicated that, compared to steel and concrete, wood-frame floor systems offer the following savings based on a 32-by-32-foot grid:
- Orlando, Florida: $4/square foot
- Los Angeles: $4/square foot
- Washington, D.C.: $3/square foot
- Houston: $3/square foot
- Charlotte, North Carolina: $2/square foot
- San Francisco: $2/square foot
Options for classroom floor assemblies include I-joists and parallel chord trusses. (Dimension lumber is suitable for the smaller spans needed for corridor assemblies.) As shown in Figure 7, the required wood member sizes for a standard classroom design are similar to steel. The table is shown over a typical grid that includes columns in the exterior walls and corridor walls, all directly aligned with classroom separation walls.
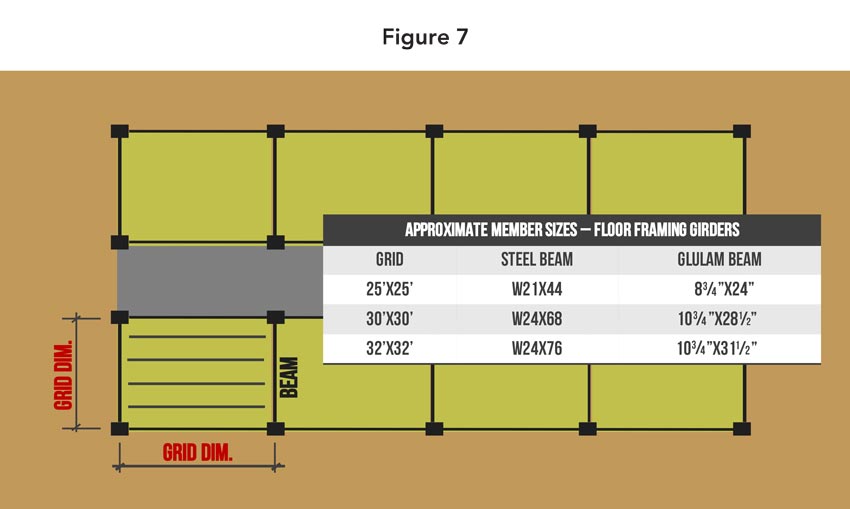
Source: WoodWorks
Assumptions: Live load = 40 psf, wood dead load = 30 psf, steel dead load = 70 psf. Sizes shown are for illustration purposes. All member sizes should be provided by a project’s structural engineer.
While Figure 7 indicates that the glulam beams are typically 3 to 71/2 inches deeper than the steel beams, this difference could be 21/2 inches smaller than the beam depths would indicate. This is because the common steel option is an open-web joist, which includes a joist seat that drops the beam by about 21/2 inches. Glulam beams can be flushed to the top of the I-joist or truss, and a top flange hanger can be used to support the truss or joist from the beam.
Although the typical grid shown in Figure 7 can be accommodated with wood framing, taking a slightly different approach can reduce costs even further. For example:
- A designer may choose not to align columns in the exterior and corridor walls with the classroom separation walls, but rather shorten the floor spans by using a column spacing (along the corridor and exterior walls) of 20 to 24 feet. Although this would likely add an additional row of columns and beams, it would reduce their size and provide shallower floor joist members, allowing more room for mechanical, electrical, and plumbing in a dropped ceiling application. Column locations would need to be coordinated with openings in the exterior and corridor walls.
- By using wood-frame corridor walls and exterior walls as bearing walls, columns and beams could be eliminated. (See Wall Framing below.)
Although not a code criteria, floor design is often governed by vibration at the span ranges above, regardless of material. Options for floor vibration analysis include the use of higher deflection criteria to add stiffness (e.g., L/480 or L/600 instead of L/360 for live load), and proprietary assemblies that have been tested and rated for vibration. A research organization, FPInnovations, has also devised a method for evaluating complete floor systems (including solid sawn joists, I-joists, parallel chord trusses, sheathing, toppings, etc.) and calculating vibration performance.
Wall Framing
Options for wall framing include solid sawn and finger-jointed dimension lumber, glulam framing, and structural composite lumber (SCL) products.
It is common in steel and concrete buildings to frame walls, both interior and exterior, with non-load bearing studs. In a wood-frame school, it can be beneficial both from a construction schedule and cost perspective to frame all walls with wood, making them load bearing where necessary for structural purposes.
In a typical steel-frame school, the building’s lateral stability against wind and seismic forces is usually provided by steel braced or moment frames or masonry shear walls. These systems may only be present in the building for the purpose of lateral load resistance. However, in a wood-frame school utilizing wood walls covered with sheathing, such as plywood or OSB, these walls can double as both gravity force-resisting members (bearing walls) and lateral force-resisting members (shear walls).
Wood-frame shear walls offer the advantages of light weight and ductility. For contrast, a typical masonry shear wall in a school might include 8-inch masonry walls with grout and reinforcing steel at 32 inches on center (o.c.). This combination has an average weight of 47 psf. A typical wood-frame shear wall in a school would be 2-by-6 studs at 16 inches o.c. with a layer of 1/2-inch plywood or OSB. This combination has an average weight of 12 psf.8
As noted, a building’s seismic forces are directly tied to its mass, meaning that the seismic forces contributed by 8-inch masonry walls would be nearly four times greater than those of the wood-frame walls. Seismic forces on a building are also directly tied to the code-specified seismic response coefficient (R). As the R term is in the denominator of the seismic force equation, a larger R value results in lower seismic forces. For seismic load resistance, wood-frame shear walls are classified as “light-frame (wood) walls sheathed with wood structural panels rated for shear resistance” (R = 6.5, per Table 12.2-1 of ASCE 7-16). This R value of 6.5 is greater than many steel and masonry lateral load-resisting systems, resulting in further reduction of seismic forces.
Tall Walls
It’s common for schools to require ‘tall walls’—20 feet and taller—to achieve desired interior heights for areas such as gymnasiums and cafeterias. Wood is both appropriate and effective in these applications.
Wood-frame tall walls offer the same benefits as other wood stud walls:
- They’re able to resist snow loads on the roof and wind loads on the wall, without requiring an additional load-bearing frame.
- When wood sheathing is added to studs, the wall is effective at resisting the lateral racking loads caused by high-wind and seismic events.
- They can be easily insulated to provide excellent thermal resistance.
- They can be finished with a wide range of finishing materials.
For these spaces, larger lumber sizes and engineered wood products can be used to obtain the same strength for walls that are taller and longer. Shear walls and connections can be easily designed to provide the required lateral resistance. Thermal requirements can be achieved with insulation. And, by paying attention to details and selecting appropriate finishing materials, tall stud walls can meet or exceed stringent fire separation requirements.
Energy Efficiency
For the Bethel School District, energy efficiency is an objective because of the cost savings. However, it underscores wood’s benefits from a thermal performance perspective.
Between 2004 and 2011, the district reduced its energy use by more than 7.6 million kilowatts and saved $4.3 million in utility costs—equivalent to the cost of electricity for 15 of its elementary schools for one year. It reported an 81 percent ENERGY STAR rating overall, and several of its 17 elementary and six junior high schools had a rating of between 95 and 98 percent. All of these schools are wood-frame.
Wood-frame building enclosures are inherently more efficient than steel-frame, concrete, or masonry construction—because of the insulating qualities of the wood structural elements, including studs, columns, and beams, and because wood stud walls are easy to insulate.9 Options also exist for insulating wood-frame buildings that aren’t available for other construction types. For example, while requirements for lighting systems or mechanical systems do not change based on structural material, wood’s versatility related to building envelope configuration gives designers more insulation flexibility.
Continuous insulation is often specified as a stand-alone prescriptive requirement or, alternatively, in conjunction with nominal insulation (e.g., between wood studs) in order to achieve higher effective R-values. Continuous insulation is necessary in structural systems using concrete and steel, which have high rates of thermal bridging, but is often avoidable in wood-frame envelopes.10
Environmental Performance
School boards, whether they receive funding from public or private sources, often include environmental performance in their objectives for school design.
In addition to the fact that wood grows naturally and is renewable, wood has a lighter carbon footprint than other common building materials.
As trees grow, they absorb carbon dioxide from the atmosphere, storing the carbon in their wood, roots, leaves or needles, and surrounding soil, and releasing the oxygen back into the atmosphere. When trees start to decay, or when the forests succumb to wildfire, insects, or disease, the stored carbon is also released. However, when trees are harvested and manufactured into products, the products continue to store much of the carbon. In the case of wood buildings, this carbon is kept out of the atmosphere for the lifetime of the structure, or longer if the wood is reclaimed and manufactured into other products. In any of these cases, the carbon cycle begins again as the forest regenerates and young seedlings once again begin absorbing carbon dioxide.
The fact that manufacturing wood into products requires less energy than other materials (and very little fossil fuel energy) also contributes to its relatively light carbon footprint.11
Life-cycle assessment (LCA) studies consistently show that wood outperforms other materials in terms of embodied energy, air and water pollution, and global warming potential.12 LCA is an internationally recognized method of evaluating the environmental impacts of materials over their life cycles, from extraction or harvest of raw materials through manufacturing, transportation, installation, use, maintenance, and disposal or recycling. It is increasingly being integrated into green building rating systems as a way to compare the impacts of alternate building designs.
Health and Well-Being
Most people in North America spend approximately 90 percent of their time indoors, either at home, at work, or in other spaces like retail stores, restaurants, schools or other public buildings. Because we spend so much time indoors, the spaces we inhabit can affect the way we act and feel, and even our health and wellbeing.
Interior design may prove to be just as important as diet, sleep habits or exercise routine. This is the premise behind biophilic design – the idea that incorporating natural elements into buildings, such as exposed wood, natural light or plants, can actually improve overall health. Research on the effects of wood as an interior element of classroom design is a relatively new area of study. In Japan, government officials have found that the use of wood in schools has a positive impact on students. The Japanese Wood Academic Society conducted a three-year study of 700 schools and reported reduced incidence of influenza outbreaks in schools featuring wood interiors vs. ``non-wood” schools.14
A one-year Austrian study observed 36 high school students, aged 13-15, who attended either fully wooden furnished classrooms or standard classrooms with plastic equipment and plasterboard walls. By the end of the year, students who were taught in wood-based environments daily had significantly lower stress levels, blood pressure and heart rates, as well as increased productivity compared to the opposite group of teenagers who didn’t have contact with wooden items. (Kelz, C. & Moser, M. 2011).15
As green building objectives have come to embrace human health issues, researchers are
increasingly looking to quantify the impact of the built environment on occupant well-being.
The results may hold promise for healthcare facilities, schools and offices to improve
performance, productivity, and occupant well-being.
Conclusion
If there is a generalization to be made about the design of educational facilities, it is that architects are often called upon to achieve many objectives with limited budgets. This may be wood’s greatest strength in the context of schools—that it typically costs less, while performing structurally and offering benefits that cover the gamut from design flexibility to carbon footprint to occupant well-being. This may also be the reason we see more wood schools over the next decade, as U.S. designers seek to satisfy the needs of a growing student population.
End Notes
1spaces4learning 2020 Facilities Construction Brief, https://spaces4learning.com/research/2019/01/facilities-construction-brief/asset.aspx?tc=assetpg
2National Center for Education Statistics, Projections of Education Statistics to 2024, http://nces.ed.gov/pubs2016/2016013.pdf
3Case Study: Bethel School District, WoodWorks, http://www.woodworks.org/wp-content/uploads/CS-Bethel2.pdf
4Case Study: El Dorado High School, WoodWorks, http://www.woodworks.org/wp-content/uploads/CS-El-Dorado.pdf
5Acoustical Considerations for Mixed-Use Wood-Frame Buildings, WoodWorks, http://www.woodworks.org/wp-content/uploads/Acoustics_Solutions_Paper.pdf, Acoustics and Mass Timber: Room-to-Room Noise Control and its accompanying Inventory of Acoustically-Tested Mass Timber Assemblies https://www.woodworks.org/wp-content/uploads/wood_solution_paper-MASS-TIMBER-ACOUSTICS.pdf, Acoustically-Tested-Mass-Timber-Assemblies-WoodWorks.pdf
62015 National Design Specification® (NDS®) for Wood Construction, Section 2.3.2.1, American Wood Council
7ASC Steel Deck Floor Deck Catalogue, http://www.ascsd.com/files/floordeck.pdf
8ASCE 7-16: Minimum Design Loads for Buildings and Other Structures, Table C3.1-1a
9Guide for Designing Energy-Efficient Building Enclosures for Wood-Frame Multi-Unit Residential Buildings in Marine to Cold Climates in North America, 2013, FPInnovations
102018 International Energy Conservation Code, Table 402.1.3
11A Synthesis of Research on Wood Products and Greenhouse Gas Impacts, FPInnovations, 2010
12Werner, F. and Richter, K., Wooden building products in comparative LCA: A literature review; International Journal of Life Cycle Assessment, 12(7):470-479, 2007
13Back to Nature: Can wood construction create healthier, more productive learning environments, Building Design + Construction, 2005
14Back to Nature: Can wood construction create healthier, more productive learning environments?
15Wood, Housing, Health, Humanity. Page 6.
![]() |
Think Wood is a leading education provider on the advantages of using softwood lumber in commercial, community and multifamily building applications. We introduce innovators in the field to our community of architects, engineers, designers and developers. For support or resources, contact us at info@ThinkWood.com. |
![]() |
WoodWorks provides education and free technical support related to the design, engineering and construction of commercial and multi-family wood buildings in the U.S. A non-profit staffed with architects, structural engineers and construction experts, we have the expertise to assist with all aspects of wood building design, including: allowable heights and areas/construction types, structural detailing of wood and hybrid systems, fire resistance and acoustical-rated assemblies, efficient and code-compliant lateral system design, alternate means of code compliance, and energy-efficient detailing. For assistance with a project, email help@woodworks.org |